
Postural activity of the diaphragm is reduced in humans when respiratory demand increases
Paul W. Hodges, Inger Heijnen and Simon C. Gandevia
Prince of Wales Medical Research Institute and University of New South Wales,
Sydney, Australia
(Received 27 April 2001; accepted after revision 29 August 2001)
- Respiratory activity of the diaphragm and other respiratory muscles is normally co-ordinated with their other functions, such as for postural control of the trunk when the limbs move. The integration may occur by summation of two inputs at the respiratory motoneurons. The present study investigated whether postural activity of the diaphragm changed when respiratory drive increased with hypercapnoea.
- Electromyographic (EMG) recordings of the diaphragm and other trunk muscles were made with intramuscular electrodes in 13 healthy volunteers. Under control conditions and while breathing through increased dead-space, subjects made rapid repetitive arm movements to disturb the stability of the spine for four periods each lasting 10 s, separated by 50 s.
- End-tidal CO2 and ventilation increased for the first 60–120 s of the trial then reached a plateau. During rapid arm movement at the start of dead-space breathing, diaphragm EMG became tonic with superimposed modulation at the frequencies of respiration and arm movement. However, when the arm was moved after 60 s of hypercapnoea, the tonic diaphragm EMG during expiration and the phasic activity with arm movement were reduced or absent. Similar changes occurred for the expiratory muscle transversus abdominis, but not for the erector spinae. The mean amplitude of intra-abdominal pressure and the phasic changes with arm movement were reduced after 60 s of hypercapnoea.
- The present data suggest that increased central respiratory drive may attenuate the postural commands reaching motoneurons. This attenuation can affect the key inspiratory and expiratory muscles and is likely to be co-ordinated at a pre-motoneuronal site.
The central nervous system co-ordinates the motor activities of all trunk muscles, including the diaphragm, during both postural and respiratory tasks. The rhythmic drive to the inspiratory motoneurons from the respiratory centres in the pons and medulla must be integrated with the other non-respiratory functions that can stop or interfere with normal respiration, such as swallowing, coughing and expulsive efforts. In addition, modulation of intra-abdominal pressure occurs through co-ordinated activity of the diaphragm, abdominal and pelvic floor muscles, and such modulation may be important for the control of spinal stability (Hodges et al. 1997; Hodges & Gandevia, 2000a).
While respiration may be interrupted during brief activities such as vomiting (Grelot & Miller, 1994), coughing (Shannon et al. 1997) and lifting (Hemborg et al. 1985), other sustained tasks must be undertaken concurrently with respiration. Recent evidence confirms that, under normal conditions, the respiratory and postural functions of the diaphragm can be co-ordinated when the stability of the trunk is challenged repetitively by repeated rapid movements of an arm (Hodges & Gandevia, 2000a). In this task, electromyographic activity (EMG) in the costal diaphragm was modulated by both respiration and arm movements. The final output to the diaphragm behaved as if there was a summation of an inspiratory and a ‘postural’ drive at the phrenic motoneurons (Hodges & Gandevia, 2000a). Integration of postural and respiratory inputs has been identified for other respiratory muscles such as the intercostal muscles (Rimmer et al. 1995) and transversus abdominis (TrA) (Hodges & Gandevia, 2000b), but not for the erector spinae (ES) or superficial abdominal muscles (Hodges & Gandevia, 2000b).
Whether this co-ordination of two drives to the phrenic motoneurons is affected by external factors, such as increased respiratory demand due to exercise or an increased chemical drive to breath, has not been established. If these two drives are simply summed, then both components should be present when either the demand for postural control or respiration is increased. However, if the activity associated with one function is abolished or reduced by increased demand for the other, this would suggest a more complex organization.
The aim of the present study was to test whether the postural activation of the diaphragm changes when respiratory demand increases with hypercapnoea and the postural perturbation produced by limb movement stays the same. Additional aims were to determine whether a major postural and respiratory muscle, TrA, responded in the same manner to hypercapnoea, and whether there was an effect on the mechanical output of these muscles, i.e. intra-abdominal pressure.
METHODS
Subjects
Recordings were made from 13 healthy volunteers (10 males and three females) who had no respiratory or neurological disease and no history of lower back pain. The means ± S.D. of age, height and weight of the subjects were 30 ± 8 years, 176 ± 6 cm and 71 ± 9 kg, respectively. Diaphragm electromyographic (EMG) recordings were attempted in nine subjects. All procedures were approved by the institutional Medical Research Ethics Committee and were in accordance with the Declaration of Helsinki. Subjects provided informed written consent.
Electromyography
Recordings from the right costal diaphragm were made using bipolar fine-wire electrodes fabricated from multi-strand, Teflon-coated stainless-steel wire (seven-strand, 110 µm, A-M systems Inc., Carslborg, WA, USA). The two wires were gently twisted and the insulation was fused by heating. The cut ends of the wires were exposed and the tips were bent back 0.5–1mm to form a hook. The electrodes were threaded into a hypodermic needle (0.70 w 50 mm) and then inserted into the diaphragm via the seventh or eighth intercostal space in the mid-clavicular line under the guidance of real-time ultrasound imaging using a 3 MHz vector array transducer (128XP/4, Acuson, Mountain View, CA, USA) (DeTroyer et al. 1997; Hodges & Gandevia, 2000a). Approximately 0.5–1ml of lidocaine (2 % with adrenaline) was injected along the proposed path to the external surface of the diaphragm. The location of the electrode was confirmed by evaluation of the EMG signal. Electromyographic activity recorded during inspiration, but not during forced expiration through pursed lips, confirmed the electrode’s location in the diaphragm (Taylor, 1960; Hodges & Gandevia, 2000c).
Recordings from the right TrA were made using bipolar fine-wire electrodes fabricated from two strands of Teflon-coated stainless- steel wire (75 µm) that were gently twisted and inserted into a hypodermic needle (38 w 0.63 mm). The Teflon coating was removed from the distal 1mm of each wire, and bent back at 1 and 2 mm to form a hook. Electrodes were inserted into TrA under ultrasound guidance midway between the anterior superior iliac spine and the distal border of the rib cage. Surface EMG electrodes (1cm diameter, Ag–AgCl discs) were placed over deltoid (anterior and posterior portions) and ES (n = 5) adjacent to the L3 spinous process. A ground electrode was placed on the right shoulder.
Successful recordings from the diaphragm were made in six subjects and from TrA in nine subjects. Diaphragm EMG data from two subjects were excluded due to the presence of movement artefacts and from one subject because of cross-talk from the internal intercostal muscle (Hodges & Gandevia, 2000c).
Respiratory measurements
Airflow was measured with a pneumotachograph and integrated on- line to be recorded as volume. Tidal volume was measured for each breath and multiplied by the instantaneous frequency to derive the minute ventilation. The mean minute ventilation was averaged for 30 s of quiet breathing, the period of arm movement (10 s) and over the 50 s of breathing between arm movement periods. End-tidal CO2 was recorded (Normocap, Datex, Helsinki, Finland) throughout the procedure, and peak values were measured and averaged for the same periods as above.
In the main trial, respiratory phase was measured using an inductance plethysmograph (Respitrace, Ambulatory Monitoring, Ardsley, NY, USA) around the chest to measure motion of the rib cage. Respiratory movements of both the rib cage and abdomen were measured in a separate trial of six subjects (including three from the main study) using bands placed around the chest and abdomen. The gains of the signals were adjusted so that the changes in amplitude of both signals were equal during an isovolume manoeuvre (Chadha et al. 1982). The relative contributions of movement of the rib cage and abdomen to tidal volume were derived from measurements of change in the rib cage and abdominal signals expressed as a percentage of the change in the sum of the two signals.
Additional recordings
Arm movement was measured with a potentiometer attached to an arm bar with its axis aligned to the approximate centre of rotation of the left shoulder. Gastric pressure (Pga) was recorded in three subjects using a pressure transducer inserted via the nose into the stomach.
Procedure
Subjects stood in a relaxed position with their feet shoulder-width apart, their arms by their sides and their left shoulder aligned to the potentiometer (Fig. 1). Baseline recordings were made while the subjects breathed quietly through the mouthpiece (volume, 170 ml) for 30 s with a nose clip. A long, flexible tube (volume, 1150 ml) was then attached to the mouthpiece to increase dead-space and induce hypercapnoea, and subjects were instructed to move their arm between 15 deg of flexion and 15 deg of extension of the shoulder, as fast as possible. Subjects were given feedback of the required range of arm movement on an oscilloscope which displayed the arm movement and the target forward and backward limits of movement. Subjects continued to breathe through the tube for 4 min during which they moved the arm for the initial 10 s of each minute (i.e. four arm movement periods of 10 s and three periods of breathing alone for 50 s). Subjects also moved their arm for 10 s in a separate trial without breathing through the increased dead-space. As there was no difference in any of the measured parameters between this condition and the initial 10 s of arm movement with the increased dead-space, only the dead-space trials are included here.
Measurements were also made of gastric pressure during the arm movement task while breathing with increased dead-space. To evaluate the relative contribution of rib cage and abdominal (diaphragm) movement to volume generation, six subjects performed an identical task to that described above with recordings of movement of both the abdomen and rib cage. This could not be undertaken in the same trial as the EMG recordings as the abdominal band of the inductance plethysmograph interfered with the abdominal EMG recordings.
Data analysis
Diaphragm EMG was filtered from 53 to 3 kHz and sampled at 5 kHz. The EMG data from the TrA, ES and Diaphragm EMG was filtered from 53 to 3 kHz and sampled at 5 kHz. The EMG data from the TrA, ES and deltoid muscles were filtered from 53 to 1kHz and sampled at 2 kHz. Pressure and rib cage movement data were sampled at 2 kHz. Data was collected using Spike2 software (Cambridge Electronic Design, Cambridge, UK) and exported for signal processing with Matlab (MathWorks, Natick, MA,USA).
The contribution of the diaphragm and other trunk muscles to respiration and postural control was assessed in two ways. Firstly, the EMG data during the movement periods were evaluated in the frequency domain to determine the power of the signal at the frequency of arm movement and the frequency of respiration. This parameter indicates the degree to which activity of each muscle changes in amplitude in conjunction with breathing or arm movement (Hodges & Gandevia, 2000a). Secondly, the EMG amplitudes during inspiration and expiration were compared to determine the extent to which the activity of each muscle was modulated during the respiratory cycle.
For the frequency analysis, the power spectral densities of the auto- correlations of the EMG and movement signals were calculated to identify the frequency of EMG bursts, and the frequency of shoulder and rib cage motion. To remove any non-stationarity from the data due to low-frequency drift, and to remove any movement artefact, the EMG data were high-pass filtered at 100 Hz (4th order zero-lag Butterworth filter), and then rectified and low-pass filtered at 30 Hz (4th order zero-lag Butterworth filter). The EMG, angular displacement of the shoulder and movement of the rib cage were then re-sampled at 1kHz so that all data were at the same sampling frequency and could be compared with identical resolution for the Fourier transform analysis. Spectral analysis was performed using a Hanning window with no overlap. Analysis of the filtered and rectified EMG signal using the ‘runs’ test indicated that the data satisfied the conditions of stationarity (Bendat & Piersol, 1971).
Analysis of the power spectral densities involved identification of the frequencies at which peaks were present that were larger than baseline noise, and the amplitude of power at the frequencies of arm movement (identified as the largest peak in the power spectrum of the arm movement data) and respiration (identified as the largest peak in the power spectrum of the rib cage data). For comparison of the amplitude of the power spectral densities at the frequencies of arm movement and respiration between periods of arm movement and between subjects, the data were normalised to the peak power recorded at the movement or respiratory frequency for that subject.
For comparison of the amplitude of diaphragm, TrA and ES EMG between inspiration and expiration, the root mean square (r.m.s.) EMG amplitude was calculated for 500 ms epochs prior to the maximum and minimum expansion of the rib cage. The baseline noise was subtracted from the r.m.s. EMG amplitude and all values were expressed as a percentage of the peak level recorded during the trial for each subject. Amplitudes were compared for three quiet breaths prior to commencement of breathing with increased dead-space, for three breaths during each period of arm movement and from the final three breaths in the 4th minute of breathing with the increased dead-space.
As measurements of gastric pressure (Pga) were made in only three subjects, analysis was limited to evaluation of parameters of Pga amplitude that were measured from the raw data. Mean Pga was measured for three breaths before movement and for the duration of each period of arm movement. Peak-to-peak variation in Pga amplitude between respiratory phases was measured for the same three quiet breaths and for three breaths during each period of arm movement. Also peak-to-peak variation in Pga amplitude was measured for five repetitions of arm movement selected at random in the middle of the movement period.
Statistical analysis
One-way repeated measures analyses of variance (ANOVAs) were used to compare the ventilatory parameters, EMG amplitudes, power of the EMG data at the movement and respiratory frequencies, mean Pga across the period of arm movement, and the peak-to-peak changes in Pga amplitude with arm movement and breathing between periods of arm movement. Duncan’s multiple range test was used for post hoc testing and the significance level was set at P < 0.05. Data are presented as means ± S.E.M. throughout the text and figures.
RESULTS
Ventilatory responses
Expected ventilatory responses to breathing with increased dead-space were recorded for all subjects. End-tidal CO2 increased from 3.4 ± 0.2 % during quiet breathing through a mouthpiece to 6.2 ± 0.2 % after breathing with increased dead-space for 1min (P< 0.001, Fig. 2B). End-tidal CO2 was not further increased during subsequent minutes of breathing with increased dead-space (Fig. 2B). Ventilation increased from 15.1 ± 1.5 l min_1 to between 32.3 ±
2.0 l min_1 during the first minute and 45.0 ± 4.3 l min_1 during the 4th minute of breathing through the increased dead-space (P < 0.001). The increase in ventilation (normalised to that recorded during quiet breathing) had reached a plateau by the 3rd minute (Fig. 2A).During the periods of arm movement, the end-tidal CO2 was lower (4.5–5.6 %) than during the periods with no movement (P < 0.05; Fig. 2B). Correspondingly, the minute ventilation was higher during the arm movement
Figure 1. Experimental set-up
The subjects stood with their left arm attached to a potentiometer. Dead-space was increased by breathing through a tube. Visual feedback of the amplitude of arm movement was provided.
periods in all but the first period, in which subjects had similar ventilation to that recorded during the remainder of the first minute of breathing with increased dead- space (Fig. 2A).
The relative contribution of rib cage and abdominal movement to volume generation was recorded in separate trials (n = 6). Expansion of the rib cage contributed 64 ± 4 % of the tidal volume (range 54–79 %) when subjects were standing at rest. When ventilation increased by breathing with increased dead-space, this proportion increased significantly to 70 ± 4 % during the first
Figure 2. Change in respiratory variables with quiet breathing and breathing with increased dead-space
Mean data for each group are shown for (A) minute ventilation, (B) end-tidal CO2 and (C) proportion of rib cage relative to abdominal motion during inspiration. Measurements made during periods of arm movement (1); and those made during periods of breathing without arm movement (0). Asterisks between the 1 and 0 indicate a significant difference between the two conditions, i.e. with and without arm movement, and asterisks above or below the pairs of data points indicate a difference between measurement epochs. Note the increase in minute ventilation, end-tidal CO2 and proportion of rib cage motion during hypercapnoea. Data are presented as means ± S.E.M.
minute (P < 0.01). It reached 73 ± 4 % during the fourth minute, although there was no significant difference between consecutive minutes of breathing with increased dead-space (Fig. 2C). During the periods of arm movement, the contribution of rib-cage motion to tidal volume was not different to that during the periods without arm movement (Fig. 2C). However, during the first period of arm movement, the contribution of rib cage motion to tidal volume was significantly greater compared to the subsequent periods (P < 0.01).
Electromyographic recordings
Recordings from a representative subject while breathing quietly and at the end of 4 min of increased ventilation are presented in Fig. 3. As expected, the amplitude of inspiratory activity in the diaphragm increased when ventilation increased (P < 0.001) and activity in the TrA increased during expiration (P < 0.02). Figure 4 shows recordings during the periods of arm movement in the first, second and fourth minute of breathing with increased dead-space. In conjunction with the increased ventilation (indicated by the increased amplitude and frequency of rib cage motion), the respiratory activity of the diaphragm was increased during the second and subsequent periods of arm movement. This is indicated by
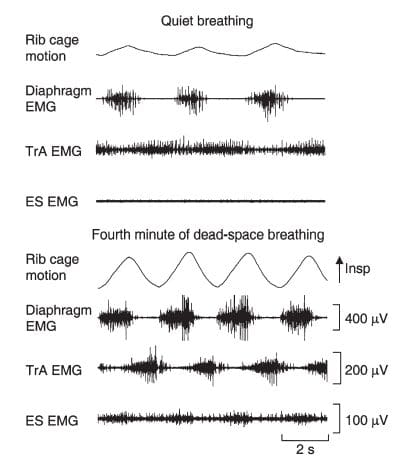
Figure 3. Electromyographic data during quiet breathing and hypercapnoea
Electromyographic (EMG) and rib-cage movement are shown for a representative subject. Note the increase in respiratory rate, diaphragm EMG during inspiration and the respiratory modulation of transversus abdominis EMG during dead-space breathing.
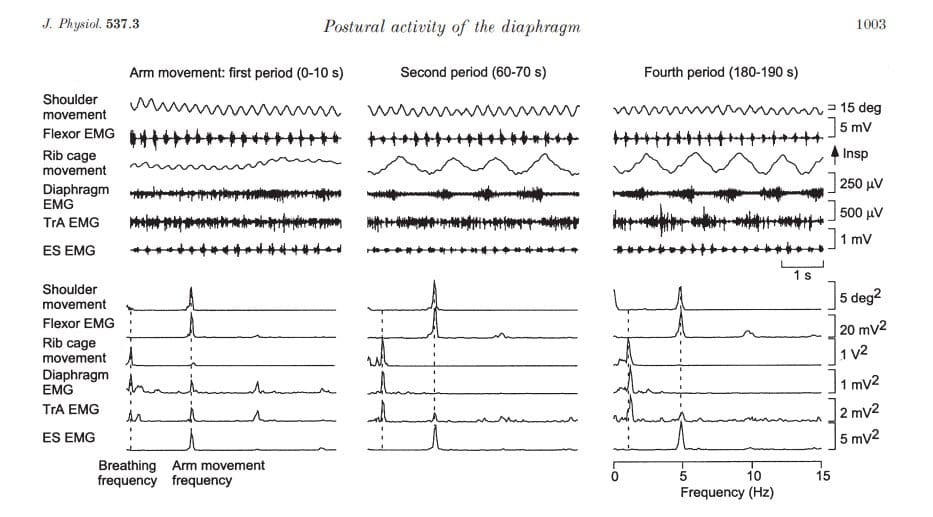
Figure 4. Raw data (upper panels) and power spectra (lower panels) for three periods of arm
movement
Data from a representative subject are shown for the first, second and fourth arm movement period. The frequencies of breathing and arm movement are indicated by the vertical dashed lines in the power spectra. The respiratory rate increased with dead-space breathing. Ventilation was increased (see increased rib-cage movement, and diaphragm and transversus abdominis electromyography (EMG)) during the second and fourth arm movement periods. The third movement period (not shown) did not differ from the second and fourth periods. By the second period of arm movement, there was no peak in the power spectrum of diaphragm EMG at the frequency of arm movement.
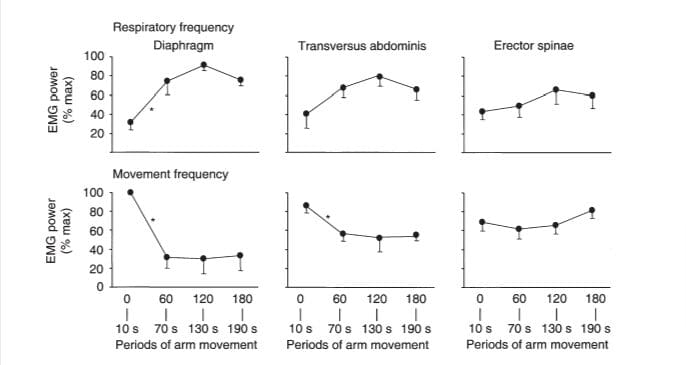
Figure 5. Electromyographic (EMG) power for three muscles at the frequencies of respiration and arm movement
The amplitude of power in the EMG spectra at the frequency of respiration (upper panels) and frequency of arm movement (lower panels) are shown. Note the reduction in power of diaphragm EMG at the frequency of arm movement in the second period of arm movement (60–70 s) and the corresponding increase in power at the frequency of respiration. A similar trend, although not significant, can be seen for transversus abdominis. * Significant difference between periods of arm movement.
the increase in power of diaphragm EMG at the frequency of respiration for this subject. This trend was confirmed for the group data, which show a significant increase in EMG power by the end of the first minute of increased ventilation (P < 0.01; Fig. 5) with no further increase during the subsequent movement periods.
The component of the diaphragm EMG associated with repetitive movement of the arm decreased in the second and subsequent periods of movement. These changes are identified in the EMG as reduced tonic activity of the diaphragm during expiration and smaller bursts of diaphragm activity with each movement of the arm (Fig. 4, upper panels). The latter change is confirmed in the power spectral densities for this subject (Fig. 4, lower panels). There was a peak in the power spectrum of the
Figure 6. Variation in the amplitude of respiratory and trunk muscle EMG with respiration during arm movements
The EMG amplitude during inspiration (0) and that during expiration (1) are shown. All amplitudes are normalised to the peak EMG recorded during the
4 min period of dead-space breathing. The EMG amplitudes during respiration at the end of the dead- space breathing are shown for comparison. Note the reduction in amplitude of diaphragm EMG during expiration in the second and subsequent arm movement periods. Erector spinae EMG increased during limb movement, but it was not modulated with respiration. * Significant differences in EMG amplitude between periods of arm movement for a specific respiratory phase.
diaphragm EMG at the frequency of arm movement in the first period of arm movement, but not in the later periods. Across the group of subjects, the power in the diaphragm EMG at the frequency of arm movement declined after 1min of increased ventilation (i.e. in the second arm movement period) (P < 0.001) and remained at that level (Fig. 5).
Group data for the amplitude of the diaphragm EMG during the last 500 ms of inspiration and expiration are presented in Fig. 6. During the initial period of arm movement, diaphragm EMG was present throughout expiration, but its amplitude was less than during inspiration, i.e. some respiratory modulation was preserved (see also Fig. 4). However, this expiratory activity declined by the second period of arm movement (P< 0.001) and remained reduced for the subsequent periods of arm movement. Throughout the trial, including the periods without arm movement, diaphragm EMG was increased during inspiration compared to quiet breathing.
Transversus abdominis EMG changed in a similar manner to that reported for the diaphragm. Figure 4 shows increased modulation of the TrA EMG with respiration after the first period of arm movement and a corresponding increase in power at the frequency of respiration. This finding is supported by the higher TrA EMG during expiration compared to inspiration (during the movement periods) for the group data (Fig. 6). In conjunction with the increased respiratory activity of TrA, its power at the frequency of arm movement decreased (Fig. 4). This reduction in power of TrA EMG with arm movement was significantly reduced after the first minute of breathing with increased dead-space (P < 0.02; Fig. 5). The changes in diaphragm and TrA EMG during the periods of arm movement with increased ventilation did not occur in the ES EMG. Erector spinae EMG power did not change at the frequencies of arm movement (P = 0.63) or respiration (P = 0.65) (Figs 4 and 5), and no respiratory modulation of ES developed during the trial.
Gastric pressure recordings
Gastric pressure was recorded in three subjects to determine whether it changed in parallel with the changes in diaphragm and TrA EMG. Data for a representative subject and the group are presented Fig. 7. Pga was maintained at an elevated level during arm movement and the mean amplitude of this Pga increase across the period of arm movement was reduced when ventilation increased (P < 0.04). In addition, the peak-to-peak variation in Pga with each arm movement was reduced when ventilation increased (P < 0.001). These findings are confirmed in the group data presented in Fig. 7B. The peak-to-peak variation in Pga between respiratory phases was greater during periods of arm movement than with quiet breathing and was increased by the third period of arm movement (120–130 s; P < 0.04; Fig. 7B).
DISCUSSION
The present data provide evidence that activity of the diaphragm associated with movement of an arm is diminished when respiratory demand is increased. Consistent with earlier studies, diaphragm activity became tonic with superimposed phasic modulation in association with respiration and arm movement at the start of dead-space breathing. However, the tonic activity and phasic modulation with arm movement were reduced or absent after only 60 s of hypercapnoea and the associated changes in Pga were reduced. Reduction in tonic and phasic activity with arm movement was also identified for an expiratory muscle, TrA. These findings suggest that increased descending respiratory drive from the pontomedullary respiratory centres may attenuate the postural activity of the diaphragm by ‘gating’ or ‘occluding’ the postural inputs to the phrenic motoneurons.
Integration of diaphragm functions
Several studies have investigated the co-ordination between the rhythmical respiratory drive to the ‘respiratory’ motoneurons from the pontomedullary centres and the other non-respiratory drives to the diaphragm. Most have investigated the modulation of respiratory drive during brief tasks such as vomiting, swallowing and coughing, which can be generated by neuronal networks in the brainstem (Grelot & Miller, 1994; Shannon et al. 1997). In tasks such as these, respiration is interrupted and the discharge of respiratory- related motoneurons is reshaped by bulbospinal neurons in the medulla, some of which may have both respiratory and non-respiratory functions (for a review, see Grelot & Bianchi, 1997). In the present study we have investigated a sustained non-respiratory task. The data at the start of the period of dead-space breathing are consistent with earlier findings that indicate respiratory activity of the diaphragm is combined with tonic and phasic changes in diaphragm EMG in association with arm movement (Hodges & Gandevia, 2000a,b). We argued that this co- ordination could reflect summation at the motoneuron pool of postural inputs from supraspinal and spinal sources with descending respiratory inputs. However, the present data suggest that this co-ordination is compromised when the chemical drive to breathe is increased. This new finding has implications for the mechanism of integration of drives to the phrenic motoneurons.
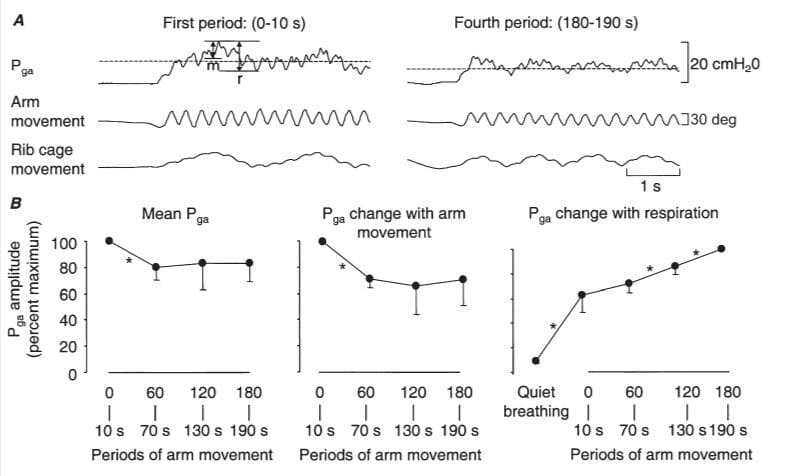
Figure 7. Pga modulation with arm movement
A, raw data from a representative subject. B, group data (n = 3) for the mean Pga and peak-to-peak amplitude of Pga with arm movement (m) and respiration (r). In A, a short period before and during arm movement is shown. The horizontal dashed line indicates the mean during the arm movement period. The mean Pga and the amplitude of the peak-to-peak changes in Pga with arm movement and respiration, expressed as a percentage of the peak Pga amplitude and averaged across the subjects, is shown in B. Note the reduction in mean Pga and peak-to-peak Pga amplitude for arm movements, and the increase in peak- to-peak Pga amplitude with respiration as respiratory demand is increased. * Significant differences between periods of arm movement.
Several organisational possibilities may explain the compromised postural activation of the diaphragm during hypercapnoea. In animals, respiration is associated with a cyclical variation in excitability of respiratory motoneurons as a result of central respiratory drive potentials (Gill & Kuno, 1963; Sears, 1964). Thus, postural input may fail to depolarise the phrenic motoneurons during expiration due to an increased inhibitory drive during this phase of respiration with hypercapnoea (Berger, 1979). The reduction of tonic and phasic diaphragm EMG in association with arm movement during expiration is consistent with this hypothesis. However, if this were the sole mechanism, bursts of EMG with arm movement would be retained during inspiration when the motoneuronal excitability is increased. This was not demonstrated. Hence, it is likely that other ‘gating’ mechanisms were contributing, presumably arising at a pre-motoneuronal level. This would explain the absence or reduction of postural activity during inspiration when motoneuronal inhibition is replaced by a facilitation. It is known that spinal proprioceptive regulation of phrenic motoneurons may be gated by the respiratory drive (Monteau & Hilaire, 1991). Morphological studies have shown pathways for pre-synaptic inhibition of primary afferents terminating on phrenic motoneurons (Goshgarian & Rafols, 1984) and weak inhibitory inputs to phrenic motoneurons are increased after removal of descending gating from the respiratory centres (Gill & Kuno, 1963). The present data are consistent with gating of the postural drive. A similar result could also occur if the postural and respiratory drives are integrated within the respiratory centres in the pons and medulla (see Shannon, 1986), or adjacent areas such as the medial reticular formation which has single descending projections to both inspiratory and expiratory motoneurons (Billig et al. 2000). Furthermore, if the respiratory and postural drives pass via interneurons, the postural drive may be occluded within this pre-motoneuronal population.
Alternatively, the absence of modulation of diaphragm EMG with arm movement during inspiration might reflect properties of the phrenic motoneuron pool. For example, if all diaphragm motoneurons were recruited maximally during inspiration, this would exclude the opportunity to recruit additional units for postural function. This is unlikely given their firing rates during voluntary tasks (Butler et al. 1999) and during similar dead-space breathing to that used here (Gandevia et al. 1999).
Functional implications
To maintain homeostasis, the CNS must prioritise respiratory drive over other functions of the respiratory muscles, such as postural control. The separate demands on the diaphragm to control pressures in the thorax (for respiration) and abdomen (for stabilisation of the lumbar spine), and for respiration via the zone of appostion (Loring & Mead, 1982) can be combined, but when the chemical drive for respiration increased, the component of diaphragm EMG associated with postural control declined. Although measurements of spinal motion are required to confirm that control of intervertebral motion of the spine was compromised in this situation, the changes in Pga recorded during arm movement with hypercapnoea are consistent with this proposal. Intra- abdominal pressure (measured here as Pga) contributes to the control of spinal stability (Bartelink, 1957; Cresswell et al. 1992; Hodges et al. 2001). As the mean amplitude of Pga and its modulation with arm movement were reduced during movements with hypercapnoea, this would suggest a reduced contribution to spinal control. Other authors have identified compromised spinal loading during lifting with hypercapnoea (McGill et al. 1995). In contrast, there are situations when respiration may be compromised. This generally involves brief tasks such as vomiting, coughing and swallowing (see above). In terms of postural control, respiration is compromised during lifting (Hemborg et al. 1985) and maximal speed is reduced by inspiration in elite athletes (Francis, 1997).
Other mechanisms may compensate for the reduced contribution of the diaphragm (and TrA) to postural control. For instance, the activity of other trunk muscles may be increased. The superficial abdominal and ES muscles contract phasically with repetitive arm movement (Zedka & Prochazka, 1997), but with no respiratory modulation (Hodges & Gandevia, 2000b; see also Fig. 6). The present data indicate that activity of ES related to arm movement was not compromised by increased respiratory demand, but the CNS does not compensate for the potential compromise of spinal control by increasing activation of this muscle. The reduced contribution of abdominal motion to inspiratory volume after breathing with increased dead-space for 60 s may be caused by increased stiffness of the abdomen as a result of enhanced recruitment of other abdominal muscles, or reduced activation of diaphragm. However, hypercapnoea leads to relatively more activation of scalene and parasternal muscles initially (Gandevia et al. 1999) which may increase the relative contribution of the rib-cage motion. Thus, postural activity of the diaphragm may be less important when respiratory demand is increased if, for example, the stiffness of the abdomen and/or thorax is increased. These alternatives remain to be determined.
Other strategies to compensate for reduced postural activity of the respiratory muscles may involve entrainment of respiration to the movement task. This occurs in numerous animals, including quadrupeds (e.g. cats (Iscoe, 1981); horses (Bramble & Carrier, 1983) and birds (e.g. Canada geese, Funk et al. 1992)). Breathing is entrained in human gait and cycling at self-selected speeds (Bramble & Carrier, 1983; Bernasconi & Kohl, 1993). Entrainment in novel tasks, such as rapid repetitive arm movement in the present study, has not been investigated in humans. However, this may simplify the co-ordination of postural and respiratory tasks by timing the respiratory recruitment of the diaphragm to specific phases in the movement task.
The findings of the present study have implications for the organisation of postural and respiratory activities of ‘respiratory’ trunk muscles and suggest that stability of the spine may be compromised in situations in which respiratory demand is increased, such as exercise and respiratory disease. Although investigation of spinal mechanics is required to confirm the extent to which spinal control is compromised by the increase in respiratory demand, it is hypothesised that such a compromise may lead to increased potential for injury to spinal structures and reduced postural control. During strenuous exercise, when the physical stresses to the spine are greater, the physiological vulnerability of the spine to injury is likely to be increased.
BARTELINK, D. L. (1957). The role of intra-abdominal pressure in relieving the pressure on the lumbar vertebral discs. Journal of Bone and Joint Surgery 39B, 718–725.
BENDAT, J. S. & PIERSOL, A. G. (1971). Random Data: Analysis and Measurement Procedures. John Wiley & Sons Inc., Chichester.
BERGER, A. J. (1979). Phrenic motoneurons in the cat: sub- populations and nature of respiratory drive potentials. Journal of Neurophysiology 42, 76–90.
BERNASCONI, P. & KOHL, J. (1993). Analysis of co-ordination between breathing and exercise rhythms in man. Journal of Physiology 471, 693–706.
BILLIG, I., FORIS, J. M., ENQUIST, L. W., CARD, J. P. & YATES, B. J.
(2000). Definition of neuronal circuitry controlling the activity of phrenic and abdominal motoneurons in the ferret using recombinant strains of pseudorabies virus. Journal of Neuroscience 20, 7446–7454.
BRAMBLE, D. M. & CARRIER, D. R. (1983). Running and breathing in mammals. Science 219, 251–256.
BUTLER, J. E., MCKENZIE, D. K. & GANDEVIA, S. C. (1999). Discharge
properties and recruitment of human diaphragmatic motor units during voluntary inspiratory tasks. Journal of Physiology 518, 907–920.
CHADHA, T. S., WATSON, H., BIRCH, S., JENOURI, G. A., SCHNEIDER,
A. W., COHN, M. A. & SACKNER, M. A. (1982). Validation of
respiratory inductive plethysmography using different calibration procedures. American Review of Respiratory Disease 125, 644–649.
CRESSWELL, A. G., GRUNDSTROM, H. & THORSTENSSON, A. (1992).
Observations on intra-abdominal pressure and patterns of abdominal intra-muscular activity in man. Acta Physiologica Scandinavica 144, 409–418.
DETROYER, A., LEEPER, J. B., MCKENZIE, D. K. & GANDEVIA, S. C.(1997). Neural drive to the diaphragm in patients with severe COPD. American Journal of Respiration and Critical Care Medicine 155, 1335–1340.FRANCIS, C. (1997). Training for Speed. Faccioni Speed and Conditioning Consultants, Canberra.
FUNK, G. D., MILSOM, W. K. & STEEVES, J. D. (1992). Coordination
of wingbeat and respiration in the Canada goose. I. Passive wing flapping. Journal of Applied Physiology 73, 1014–1024.
GANDEVIA, S. C., GORMAN, R. B., MCKENZIE, D. K. & DE TROYER, A.
(1999). Effects of increased ventilatory drive on motor unit firing rates in human inspiratory muscles. American Journal of Respiration and Critical Care Medicine 160, 1598–1603.
GILL, P. K. & KUNO, M. (1963). Excitatory and inhibitory actions on phrenic motoneurones. Journal of Physiology 168, 274–289.
GOSHGARIAN, H. G. & RAFOLS, J. A. (1984). The ultrastructure and synaptic architecture of phrenic motor neurons in the spinal cord of the adult rat. Journal of Neurocytology 13, 85–109.
GRELOT, L. & BIANCHI, A. L. (1997). Multifunctional medullary respiratory neurons. In Neural Control of the Respiratory Muscles, ed. MILLER, A. D., BIANCHI, A. L. & BISHOP, B. P., pp. 297–304.
CRC Press, Inc., Boca Raton, FL, USA.
GRELOT, L. & MILLER, A. D. (1994). Vomitting – its ins and outs.
News in the Physiological Sciences 9, 142.
HEMBORG, B., MORITZ, U. & LÖING, H. (1985). Intra-abdominal
pressure and trunk muscle activity during lifting. IV. The causal factors of the intra-abdominal pressure rise. Scandinavian Journal of Rehabilitation Medicine 17, 25–38.
HODGES, P. W., BUTLER, J. E., MCKENZIE, D. & GANDEVIA, S. C.
(1997). Contraction of the human diaphragm during postural adjustments. Journal of Physiology 505, 239–248.
HODGES, P. W., CRESSWELL, A. G., DAGGFELDT, K. & THORSTENSSON, A.
(2001). In vivo measurement of the effect of intra-abdominal pressure on the human spine. Journal of Biomechanics 34, 347–353.
HODGES, P. & GANDEVIA, S. (2000a). Activation of the human diaphragm during a repetitive postural task. Journal of Physiology 522, 165–175.
HODGES, P. & GANDEVIA, S. (2000b). Changes in intra-abdominal pressure during postural and respiratory activation of the human diaphragm. Journal of Applied Physiology 89, 967–976.
HODGES, P. W. & GANDEVIA, S. C. (2000c). Pitfalls of intramuscular electromyographic recordings from the human costal diaphragm. Clinical Neurology 111, 1420–1424.
ISCOE, S. (1981). Respiratory and stepping frequencies in conscious exercising cats. Journal of Applied Physiology 51, 835–839.
LORING, S. H. & MEAD, J. (1982). Action of the diaphragm on the rib cage inferred from a force-balance analysis. Journal of Applied Physiology 53, 756–760.
MCGILL, S. M., SHARRATT, M. T. & SEGUIN, J. P. (1995). Loads on
spinal tissues during simultaneous lifting and ventilatory challenge. Ergonomics 38, 1772–1792.
MONTEAU, R. & HILAIRE, G. (1991). Spinal respiratory motoneurons.Progress in Neurobiology 36, 83–144.
RIMMER, K. P., FORD, G. T. & WHITELAW, W. A. (1995). Interaction
between postural and respiratory control of human intercostal muscles. Journal of Applied Physiology 79, 1556–1561.
SEARS, T. A. (1964). The slow potentials of thoracic respiratory motoneurones and their relation to breathing. Journal of Physiology 175, 404–424.
SHANNON, R. (1986). Reflexes from respiratory muscles and costovertebral joints. In Handbook of Physiology, section 3, The Respiratory System, vol II, Control of Breathing, part 1, sect. ed. FISHMAN, A. P., vol. ed. CHERNIACK, N. S. & WIDDICOMBE, J. G.,
exec. ed. GEIGER, S. R., pp. 431–447. American Physiological Society, Waverly Press, Baltimore, MD, USA.
SHANNON, R., BOLSER, D. C. & LINDSEY, B. G. (1997). Neural control
of coughing and sneezing. In Neural Control of the Respiratory Muscles, ed. MILLER, A. D., BIANCHI, A. L. & BISHOP, B. P.,
pp. 213–222. CRC Press, Inc., Boca Raton, FL, USA.
TAYLOR, A. (1960). The contribution of the intercostal muscles to the effort of respiration in man. Journal of Physiology 151, 390–402.ZEDKA, M. & PROCHAZKA, A. (1997). Phasic activity in the human erector spinae during repetitive hand movements. Journal of Physiology 504, 727–734.
Acknowledgements
We thank Dr Jane Butler for assistance with data collection and discussion of the manuscript. Financial support was provided by the National Health and Medical Research Council of Australia.
Corresponding author
P. W. Hodges: Department of Physiotherapy, The University of Queensland, Brisbane, QLD 4072, Australia.
Email: [email protected]